Decreased pH on the Calcification of Scleractinian Corals
Stressor Review
Organism
Scleractinian corals are abundant in shallow
, nutrient-poor (oligotrophic), tropical areas and live in association with zooxanthellae, or symbiotic dinoflagellates (Symbiodinium sp.). Corals precipitate aragonitic CaCO3 to produce supportive skeletons (Al-Horani et al. 2003).
The rate of calcification of a coral reef is assumed to be around 10 kgCaCO3m
-2 year
-1 (Tambutté et al. 1996).
Physiology of calcification
Corals possess a multi-compartmental structure composed of two tissue layers (oral and aboral) separated by the coelenteron. The calcifying layer (calicoblastic layer) faces the skeleton. The symbionts (zooxanthellae) are present within a perisymbiotic membrane in the endodermal cells (Al-Horani et al. 2003).
http://media-2.web.britannica.com/eb-media/94/26994-004-9E1DBF8B.jpg
Symbionts synthesize various forms of carbohydrates transported to growing parts of the polyp to generate ATP (Al-Horani et al. 2003). Cyclic photophosphorylation, a light-dependent process, supplies the required ATP for the synthesis of proteins and lipids used by calcification (Al-Horani et al. 2003).
(McConnaughey and Whelan 1997)
The deposition of the calcium carbonate skeleton by scleractinian corals, “skeletogenesis”, is a highly organized extracellular process regulated by a process that combines organic matrix and mineral depositions in seasonal to diurnal growth layers (Marubini et al. 2008).
Calcification rate is controlled by the calicoblastic epithelium, which provides peri-crystalline fluid (i.e., the fluid filling the space between epithelium and skeleton) with the organic matrix (including glycoproteins, proteins and phospholipids) and transports calcium ions and dissolved inorganic carbon (DIC) (Marubini et al. 2008).
Ca2+ and CO32_ ions obtained from seawater precipitate in the calcioblastic epithelium of the coral polyp to form crystals of the calcium carbonate mineral aragonite (Langdon and Atkinson 2005). At peak calcification rate, calicoblastic epithelium cells have been known to transport hourly all the calcium contained in 50–100 times their own volume (Tambutté et al. 1996).
http://coris.noaa.gov/about/what_are/cross_section2.jpg
(Tambutté et al. 1996)
In a study by Al-Horani et al. (2003), the mechanism of calcification and its relation to photosynthesis and respiration were studied with Ca2+, pH and O2 microsensors using the scleractinian
coral Galaxea fascicularis. Prior to this study, light was known to enhance calcification, though the mechanism remained allusive. The authors describe the mechanism of calcification in corals as follows:
“Ca-ATPase generates two gradients of Ca2+ by continuously pumping Ca2+ to the calcifying site in light and, thus, decreasing the concentration of calcium in the calicoblastic cells and the coelenteron. At the same time it increases the calcium concentration at the calcifying site. This leads to continuous diffusion of Ca2+ to the calicoblastic cells along the concentration gradient from seawater. The aragonite saturation state is increased strongly, from ca. 3.2 in the dark to ca. 25 in the light at the calcifying site. The transport of Ca2+ to the calcifying site is coupled with the transport of protons away from it, and the pH is increased to ca. 9.3, thereby increasing the carbonate concentration at the calcification site. These conditions favor high rates of calcification in the light compared with the dark. Oxidative phosphorylation of photosynthates is a main process that supplies the ATP needed to power energy requiring processes in light,” (Al-Horani et al. 2003).
(Al-Horani et al. 2003)
Al-Horani et al. conclude that light triggers calcium uptake while energy generation is necessitated for the transport of ions and synthesis of organic matrix (Al-Horani et al. 2003).
It should be noted that azooxanthellae corals have calcification rates similar to zooxanthellae corals though their mechanisms differ (Marshall 1996).
Stressor
Ocean Acidification
Global
Future projections from climate system and carbon models suggest that atmospheric CO2 concentrations by the end of the twenty-first century will be close to 700 ppmv (~370 ppmv at present) (Andersson et al. 2009).
Increased atmospheric CO2 will subsequently lead to increased dissolved inorganic carbon in the mixed layer of the ocean due to the increased uptake of gaseous CO2 in seawater according to:
CO2 + H2O + CO32- = 2HCO3-
Consequently CO32
- concentration will decrease, thereby lowering the saturation state of seawater with respect to carbonate mineral (Andersson et al. 2009).
While carbonate chemistry was generally not considered to be an important parameter controlling calcification until recently (Gattuso et al. 1999), experimental evidence now indicates that the rate of calcification of corals exhibits a strong positive correlation to saturation state (Andersson et al. 2009).
Consequently, it has been suggested that calcification by coral reefs will decrease significantly by the middle of the twenty-first century owing to increased atmospheric CO2 and subsequent decreased carbonate saturation state
(Andersson et al. 2009; Gattuso et al. 1999).
It is assumed that global change-induced ocean acidification is currently leading to a global decrease in marine calcification (Raven et al. 2005 as ctd. in Marubini et al. 2008).
Global in combination with local
Global stressors will be made manifest at the local scale, interacting with local stressors to produce a negative cumulative effect; a synergistic effect of the stressors that has a greater effect than any stressor in isolation (Rusell et al. 2009).
Notably, global and local stressors can combine to cause changes that are greater than their sum (Rusell et al. 2009).
Because stressors are typically studied in isolation, the cumulative effects of individual stressors will be underestimated (Rusell et al. 2009;
Langdon and Atkinson 2005).
Response
Organismal
Marubini et al. (2008)
studied t
he effect of seawater acidification on the calcification and photosynthesis of the scleractinian tropical coral Stylophora pistillata. They found that seawater acidification decreased coral calcification by ca. 0.1mg CaCO3 g-1 d-1 per decrease of 0.1 pH unit. According to the authors, this correlation suggests that seawater acidification affects coral calcification by decreasing the availability of the CO32- substrate for calcification. However, the authors recognized that a decrease in coral calcification could also be attributed to either a decrease in extra- or intracellular pH, or to a change in the buffering capacity of the medium, impairing supply of CO32- from HCO3- (Marubini et al. 2008).
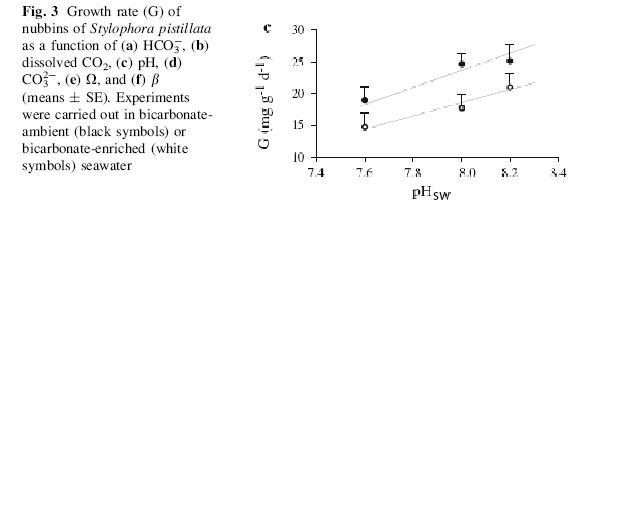
(Marubini et al. 2008)
As Marubini et al. (2008) found coral growth to be correlated with three parameters (pH, CO3 2- and CO2), they establish 5 hypothesis for the related mechanism :
(1) Seawater acidification affects coral calcification through a decrease in photosynthesis.
Since the authors did not find that calcification and photosynthesis were correlated, this hypothesis was not supported by the results of this study.
(2) Seawater acidification affects coral calcification through a decrease in carbonate concentration.
This hypothesis is supported by the significant correlation between growth and CO32- concentration and is in agreement with previous studies performed at the organism and community levels (e.g. Schneider and Erez 2006).
A doubling of the seawater bicarbonate concentration lead to an increase in mean growth of 27%, independent of pH conditions, suggesting that calcification was C-limited (Marubini et al. 2008).
The authors suggest u
p-regulation of proteins involved in C-transport is one explanation of these results and indicates that corals are able to adapt to changes in DIC concentration(Marubini et al. 2008).
A second explanation involves the enhancement of calcification by an enhancement of photosynthetic products, as was evinced by the increase in both photosynthesis and calcification with the addition of bicarbonate (Marubini et al. 2008).
(3) Seawater acidification affects coral calcification through an inhibition induced by high CO2 concentrations.
CO2 may lead to acidification of the medium and subsequent alteration of biological processes. However, the authors suggest that a CO2 increase would insufficiently decrease intra-and extracellular pH units, as the values would remain within the range of natural variation experienced by the organism
(4) Seawater acidification affects coral calcification through an inhibition induced by decreasing pH.
Yet, while the range of seawater pH, or extracellular pH (pHe) used in the present study (and throughout the literature) is large, it remains in the same range as those observed during a daily cycle in coral tissue or within the ecosystem, implying that coral cells are already adapted to small variations in external pH.
(5) Seawater acidification affects coral calcification indirectly through a change in the buffering capacity of the coelenteric space or of the peri-crystalline fluid.
Buffering capacity (B, expressed as mM pH units-1) is defined as: B = dB/dpH, where dB is the amount of base (or H+) added to the solution and dpH is the change in pH of the solution due tothat base (or acid) addition. Marubini et al. (2008) found an inverse relationship between coral calcification and B, suggesting that low buffering capacity of the media is associated with high rates of coral calcification.
Population
The rate of skeletal growth of corals is a major determinant of their fitness and ecological success(Langdon and Atkinson 2005). Skeletal growth determines a coral colony’s ability to compete for space and light, and its ability to repair structural damage caused by humans, storms, grazers or bioeroders(Langdon and Atkinson 2005). Large coral colonies have a greater reproductive output and a competitive advantage over smaller colonies. The rate of growth also governs how long new coral recruits and coral fragments take to reach the critical colony size for sexual maturity and start reproducing (Langdon and Atkinson 2005).
In a study of Acropora palmata, (zooxanthellae coral) Bak et al. (2009) show that the linear growth rates of coral branches from 2002–2004 were 7.2% lower in summer and 10.7% lower in winter compared with rates from 1971–1973. Recognizing possible causative environmental factors relating to these
decreases in growth rate, the authors do not dismiss the possibility that a change in ocean
pH could be responsible for the drop in branch extension rate (Bak et al. 2009).
Long Term Implications
The results of the study by Marubini et al. (2008) confirm that global change induced seawater acidification may lead to a decrease of tropical coral growth calcification. Using the average emission scenario of the Intergovernmental panel on Climate Change, Leclercq et al (2000) predict that the calcification rate of scleractinian-dominated communities may decrease by 21% between the pre-industrial period (year 1880) and the time at which
pCO2 will double (year 2065
). While the biological mechanism underlying this decrease remains to be elucidated, as growth is dependant on calcification, ocean acidification will likely have many implication on the fitness and ecological success of corals.
In the first ecosystem-scale validation of predictions that calcareous organisms are susceptible to elevated amounts of pCO2, Hall-Spencer et al. 2008 show the effects of acidification on benthic ecosystems at shallow coastal sites where volcanic CO2 vents lower the pH of the water column. Along gradients of normal pH (8.1–8.2) to lowered pH (mean 7.8–7.9, minimum 7.4–7.5), typical rocky shore communities with abundant calcareous organisms shifted to communities lacking scleractinian corals (Spencer et al. 2008). This study showed how hazardous the projected rise in atmospheric CO2 concentration is, as it is likely to bring about reductions in biodiversity and radically alter ecosystems (Hall-Spencer et al. 2008).
In addition to ocean acidification, an increase in other environmental impacts and mass bleaching in corals will likely combine to force coral reefs into rapid and terminal decline world-wide (Veron et al. 2009).
It should be noted that while studies have shown that some organisms will be able to adapt, evidence exists suggesting that organisms cannot acclimate their rates of calcification to increased CO2 (Rusell et al. 2009; Wooldridge and Done 2009).
Moreover, Andersson et al. (2009) show that
significant impact on coral reef ecosystems will occur despite seemingly promising changes to saturation state and pH restored by the dissolution of metastable carbonate minerals.
Policy
Increasing acidification is likely to increase coral vulnerability and correspondingly decrease its resistance. Not only are there implications on the health of the corals themselves, but also on the reef ecosystems they belong to and the livelihoods that depend on them. Improved coral reef management is thus imperative.
Rusell et al. (2009) empower local managers by showing that policy taken to reduce the effects of local stressors can reduce the effects of global stressors which are not under local governance; thus local management may be effective in reducing the effects of climate change (Rusell et al. 2009).
Management options as related to calcification have been alluded to in the literature as well.
Results showing that the negative effect of acidification may be counteracted by increasing the bicarbonate concentration of seawater have been described by Marubini et al. 2008.
Whether or not this is a feasible management strategy has yet to be determined.
There exists however, promising evidence for the increase of regional-scale survival prospects of coral reefs to global climate change. In a study investigating geographic patterns of coral bleaching on the Great Barrier Reef, Australia, Wooldridge and Done (2009) report on coral reef management actions that have successfully mitigated climate change effects on coral reefs by causing nutrients to become limiting to proliferation of benthic algae. One can only hope that these results like these will lead to more proactive management.
References
Al-Horani F, SM Al- Moghrabi and D. de Beer.
2003.
The mechanism of calcification and its relation to photosynthesis and respiration in the scleractinian coral
Galaxea fascicularis.
Marine Biology.
142: 419-426.
Andersson AJ, FT Mackenzie and LM Ver.
2003.
Solution of shallow-water carbonates: An insignificant buffer against rising atmospheric CO2.
Geology.
31: 513-516.
Bak RPM, G Nieuwland and EH Meesters.
2009.
Coral growth rates revisited after 31 years: What is causing lower extension rates in
Acropora palmate?
Bulletin of Marine Science.
84(3): 287-294.
Feely RA, CL Sabine, K Lee, W Berelson, J Kleypas, VJ Fabry and FJ Millero.
2004.
Impact of anthropogenic CO2 on the CaCO3 system in the oceans.
Science.
305: 362-366.
Gattuso JP, D Allemand and M Frankignoulle.
1999.
Photosynthesis and calcification at cellular, organismal and community levels in coral reefs:
A review on interactions and control by carbonate chemistry.
American Zoologist.
39(1); 160-183.
Hall-Spencer JM, R Rodolfo-Metalpa, S Martin, E Ransome, M Fine, SM Turner, SJ Rowley, D Tedesco and MC Buia.
2008.
Volcanic carbon dioxide vents show ecosystem effects of ocean acidification.
Nature.
454: 96-99.
Langdon C and MJ Atkinson. 2005. Effect of elevates pCO2 on photosynthesis and calcification of corals and interactions with seasonal change in temperature/irradiance and nutrient enrichment. Journal of geophysical research. 110: C09S07.
Leclercq N, JP Gattuso and J Jaubert. 2000. CO2 partial pressure controls the calcification rate of a coral community. Global Change Biology. 6: 329-334.
Marshall AT. 1996. Calcification in hermatypic and ahermatypic corals. Science. 271(5249): 637-639.
Marubini F, C Ferrier-Pagès, P Furla and D Allemand. 2008. Coral calcification responds to seawater acidification: working hypothesis towards a physiological mechanism. Coral Reefs. 27: 491-499.
McConnaughey TA and JF Whelan. 1997. Calcification generates protons for nutrient and bicarbonate uptake. Earth-Science Reviews. 42: 95-117.
Raven J, K Caldeira, H Elderfield, O Hoegh-Guldberg, P Liss, U Riebesell, J Shepherd, C Turley and A Watson. 2005. Ocean acidification due to increasing atmospheric carbon dioxide. Policy Document 12/05. Royal Society, London.
Russell BD, JI Thompson, LJ Falkenberg and SD Connell. 2009. Synergistic effects of climate change and local stressors: CO2 and nutrient-driven change in subtidal rocky habitats. Global Change Biology. 15: 2153-2162.
Schneider K and J Erez. 2006. The effect of carbonate chemistry on calcification and photosynthesis in the hermatypic coral Acorpora eurystoma. Journal of Limnology and Oceanography. 51(3): 1284-1293.
Tambutté É, D Allemand, E Mueller and J Jaubert. 1996. A compartmental approach to the mechanism of calcification in hermatypic corals. Journal of Experimental Biology. 199: 1029-1041.
Veron JEN, O Hoegh-Guldberg, TM Lenton, JM Lough, DO Obura, P Pearce-Kelly, CRC Sheppard, M Spalding, MG Stafford-Smith, AD Rogers.
2009.
The coral reef crisis: The critical importance of <350 ppm CO2.
Marine Pollution Bulletin.
58: 1428-1436.
Wooldridge SA and TJ Done.
2009.
Improved water quality can ameliorate effects of climate change on corals.
Ecological Applications.
19(6): 1492-1499.
This page was developed as part of the course at the University of Washington: Integrative Environmental Physiology